Aneeqa Khan, Manchester University
Nuclear fusion is the process that powers the Sun and all other stars. During fusion, the nuclei of two atoms are brought close enough together that they fuse together, releasing huge amounts of energy.
Replicating this process on Earth has the potential to deliver almost limitless electricity with virtually zero carbon emissions and greater safety, and without the same level of nuclear waste as fission.
But building what is essentially a mini star on Earth and holding it together inside a reactor is not an easy task. It requires immense temperatures and pressures and extremely strong magnetic fields.
Right now we don’t quite have materials capable of withstanding these extremes. But researchers like me are working to develop them, and we’ve found some exciting things along the way.
Tokamaks
There are many ways to contain nuclear fusion reactions on Earth, but the most common uses a doughnut shaped device called a tokamak. Inside the tokamak, the fuels for the reaction – isotopes of hydrogen called deuterium and tritium – are heated until they become a plasma. A plasma is when the electrons in the atoms have enough energy to escape the nuclei and start to float around. Because it’s made up of electrically charged particles, unlike a normal gas, it can be contained in a magnetic field. This means it doesn’t touch the reactor sides – instead, it floats in the middle in a doughnut shape.
When deuterium and tritium have enough energy they fuse together, creating helium, neutrons and releasing energy. The plasma has to reach temperatures of 100 million degrees Celsius for large amounts of fusion to happen – ten times hotter than the centre of the Sun. It has to be much hotter because the Sun has a much higher density of particles.
Although it’s mostly contained within a magnetic field, the reactor still has to withstand huge temperatures. At Iter, the world’s biggest fusion experiment, expected to be built by 2035, the hottest part of the machine would reach around 1,300℃.
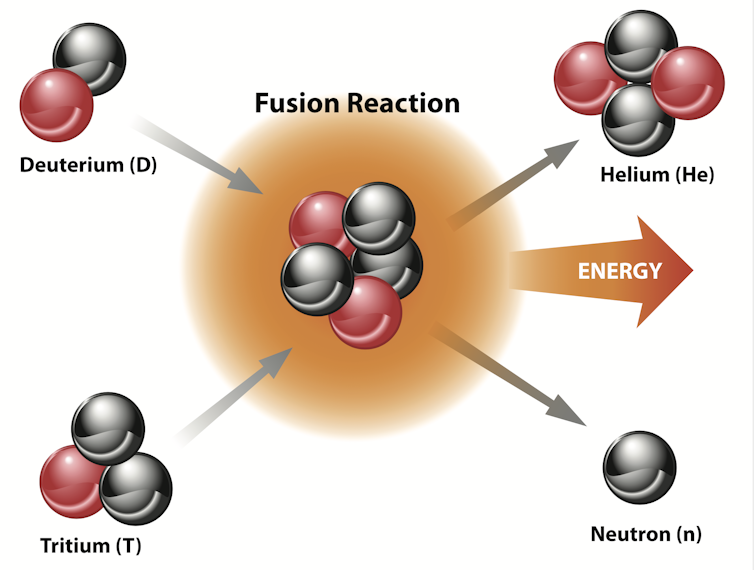
While the plasma will mostly be contained in a magnetic field, there are times when the plasma might collide with the walls of the reactor. This can result in erosion, fuel being implanted in the walls and modifications to the material properties.
On top of the extreme temperatures, we also have to consider the by-products of the fusion reaction of deuterium and tritium, like extremely high energy neutrons. Neutrons have no charge so can’t be contained by the magnetic field. This means they hit against the walls of the reactor, causing damage.
The breakthroughs
All these incredibly complex challenges have contributed to huge advances in materials over the years. One of the most notable has been high temperature superconducting magnets, which are being used by various different fusion projects. These behave as superconductors at temperatures below the boiling point of liquid nitrogen. While this sounds cold, it’s high compared to the much colder temperatures other superconductors need.
In fusion, these magnets are only metres away from the high temperatures inside the tokamak, creating an enormously large temperature gradient. These magnets have the potential to generate much stronger magnetic fields than conventional superconductors, which can dramatically reduce the size of a fusion reactor and may speed up the development of commercial fusion.
We do have some materials designed to cope with the various challenges we throw at them in a fusion reactor. The front-runners at the moment are reduced activation steels, which have an altered composition to traditional steels so the levels of activation from neutron damage is reduced, and tungsten.
One of the coolest things in science is something initially seen as a potential issue can turn into something positive. Fusion is no exception to this, and one very niche but noteworthy example is the case of tungsten fuzz. Fuzz is a nanostructure that forms on tungsten when exposed to helium plasma during fusion experiments. Initially considered a potential issue due to fears of erosion, there’s now research into non fusion applications, including solar water splitting – breaking it down into hydrogen and oxygen.
However, no material is perfect, and there are several remaining issues. These include the manufacture of reduced activation materials at a large scale and the intrinsic brittleness of tungsten, which makes it a challenge to work with. We need to improve and refine on the existing materials we have.
The challenges
Despite the huge advances in the field of materials for fusion, there’s still a lot of work that needs to be done. The main issue is we rely on several proxy experiments to recreate potential reactor conditions, and have to try and stitch this data together, often using very small samples. Detailed modelling work helps to extrapolate predictions of material performance. It would be much better if we could test our materials in real situations.
The pandemic has had a major impact on materials research because it’s been more difficult to carry out real life experiments. It’s really important that we continue to develop and use advanced models to predict material performance. This can be combined with advances in machine learning, to identify the key experiments we need to focus on and identify the best materials for the job in future reactors.
The manufacturing of new materials has typically been in small batches, focusing only on producing enough materials for experiments. Going forward, more companies will continue to work on fusion and there will be more programmes working on experimental reactors or prototypes.
Because of this, we are getting to the stage where we need to think more about industrialisation and development of supply chains. As we edge closer to prototype reactors and hopefully power plants in the future, developing robust large scale supply chains will be a huge challenge.
Aneeqa Khan, Research Fellow in Fusion, Manchester University
This article is republished from The Conversation under a Creative Commons license. Read the original article.